Relativistic laser-plasma generation
Within the present research activity we aim at exploiting the unique possibilities offered by the combination
of cryogenic RDBs and high-power lasers to provide new insights into the bulk matter heating mechanisms in intense laser-plasma
interactions, the understanding of which represents one of the great challenges of modern relativistic laser-plasma research.
Advances in state-of-the-art laser technology have led to the development of petawatt laser systems that can generate ultra-short
pulses on the sub-picosecond (< 10-12 s) time scale. When focused to a spot size a few micrometers wide, these laser pulses can
exceed peak intensities of 1021 W cm-2, thus producing energy densities on a micrometer length-scale that are inaccessible by
any other method. The interaction of such intense light pulses with bulk matter generates extreme conditions that bring
astrophysics into the laboratory, and might provide the route to the realization of the next generation tabletop-scale
particle accelerators with potential applications in, e.g., ion-based cancer therapy.
Usually, in laser-plasma experiments thin metal foils a few micron thick are used as a target. The laser energy
is transferred to the bulk material via the production at the front side of the foil of "hot" electrons at relativistic energies,
which can then easily penetrate the target material. However, the transverse extension of the employed foil targets, which are
typically of mm2 size, lead to a substantial lateral spread of the laser-produced hot electrons, thereby precluding the heating
of the target material to very high temperatures. In addition, these experiments suffer from the major drawback that the target
foils must be replaced after each laser shot, thus precluding detailed parametric studies that require the collection of a large
amount of data and the operation of the laser-driven ion source in a (quasi-) continuous mode. In this regard, RDBs turn out to
provide nearly ideal target systems for relativistic laser-plasma applications. The droplets are delivered at MHz repetition rate
and it is known, both theoretically and experimentally, that targets with a size comparable to the laser spot size (so
called "mass-limited" targets) offer a mean to increase the absorption efficiency of the lase energy. Cryogenic materials such as
hydrogen and argon are especially attractive systems because of their scientific relevance. For example, pure mass-limited
solid-density hydrogen targets are ideally suited for the realization of a continuous laser-driven proton source. Hydrogen under
the extreme temperature and pressure conditions produced in relativistic laser-plasma interactions is of central interest in
planetary context, for example as model system for studies of the equation of state of the interior of giant planets such as
Jupiter.
Unfortunately, producing hydrogen or argon RDBs is not that easy. Owing to the high vapor pressures of liquid
argon and hydrogen the expanding filament rapidly cools below its normal melting point and freezes well before Rayleigh breakup
can take place
(click here to read more).
In our group we have devoted considerable efforts to come up with the above challenging issue, and have been recently able to
experimentally demonstrate the production of periodic argon and hydrogen droplet beams at IKF
(Costa Fraga et al., 2012). As the most important feature, our
droplet injector makes use of a glass capillary that is inserted into an outer glass capillary tube, as shown in Fig. 1a. As
the liquid jet emerges from the inner capillary it expands in an axially co-flowing gas that suppresses the jet freezing by
significantly slowing down the evaporative cooling, thus enabling the Rayleigh breakup of the continuous filament and the
subsequent injection of the droplet stream into vacuum (Figs 1b and 1c). Our design provides in particular a very compact
injection source that will greatly facilitate the droplet beam operation in a vacuum envi-ronment characterized by the
presence of many delicate optical components as typically encountered in laser-plasma generation experiments. Given the
comparatively small sizes of both the laser beam focus and of the liquid jet, alignment represents the major issue. In this
regard, we have shown that our injector allows producing periodic droplet beams that are spatially stable within few
micrometers during several hours of continuous operation at cryogenic temperatures
(Costa Fraga et al., 2012).
|
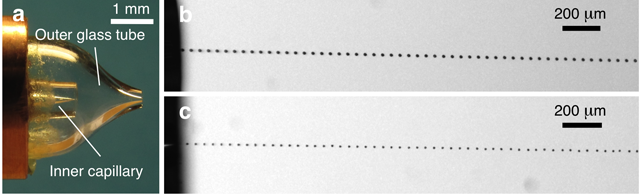
- Figure 1.
(a) Enlarged view of the exit end of our cryogenic droplet injector evidencing the outer glass tube and the central inner capillary. (b),
(c) Stroboscopic images of periodic argon (b) and (c) hydrogen droplet beams propagating in vacuum. The argon droplets are ∼ 21 μm in
diameter, whereas the hydrogen droplet diameter is ∼ 13 μm. (Adapted from
Costa Fraga et al., 2012).
|
We have explicitly demonstrated the capability of our cryogenic RDB source by performing a proof-of-principle
experiment at the PHELIX petawatt laser facility at GSI, in
collaboration with Dr. Paul Neumayer, who is fellow at the ExtreMe Matter Institute (EMMI),
and Prof. Malte C. Kaluza, who is head of one of the leading groups worldwide on laser-induced particle acceleration and detection
at the Institut für Optik und Quantenelektronik (IOQ) in Jena. During these preliminary
experiments terawatt laser pulses from the PHELIX pre-amplifier stage were focused onto argon droplets of ≈ 20 μm diameter (see Fig. 2a).
Time-integrated 2D-images of the droplet thermal emission in the XUV spectral region (Fig. 2b) indicate that the resulting plasma is
confined to the initial droplet size during the thermal emission stage, thus supporting the conclusion that the coupling of the
laser-energy is effectively confined to a small volume, providing isochoric heating of the solid density argon to very high temperatures
by using rather moderate laser energies on a time scale that is short compared to hydrodynamic motion. The inferred bulk electron
temperature of 160 eV is comparable to that obtained at significantly higher laser energies, and thus conclusively demonstrating that
RDBs represent indeed a unique target to generate matter at extreme energy densities by intense laser irradiation.
|
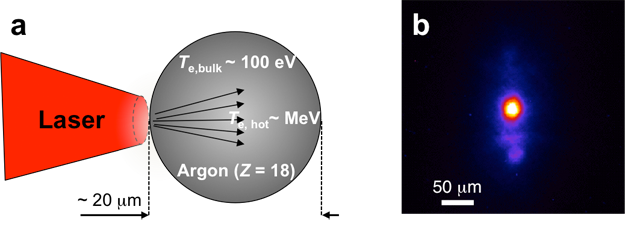
- Figure 2.
(a) Schematic view of the experiment performed at the PHELIX laser facility (
Costa Fraga et al., 2012). Plasma conditions at extreme pressures are produced through rapid isochoric heating of the droplet
material by the laser-generated relativistic electrons (represented by the arrows), which are then pushed back by the high electrostatic
sheath fields that surround the target as a result of charge separation. (b) Time-integrated XUV image of the droplet thermal emission.
The laser pulse came from the left.
|
We are currently planning within our collaboration a further series of experiments to investigate crucial issues related
to (1) laser-driven proton acceleration and (2) generation of high energy density matter. To achieve these goals we will employ pulses
from the JETI laser at IOQ and the
PHELIX laser, which are complementary with respect to both the laser
energy and repetition rate,
providing pulses at a high repetition rate, yet in the mid energy range (∼ 1 J) for the former, and at a low repetition rate but
containing up to ∼ 100 J for the latter.